Tourette’s disorder is characterized by motor and phonic tics that fluctuate in severity. Anatomical and functional disturbances of cortico-striato-thalamo-cortical circuits are presumed to play a major role in causing Tourette’s disorder symptoms
(1). These complex neural circuits include premotor, motor, and somatosensory cortices and related portions of the basal ganglia and thalamus
(2), as well as various prefrontal regions that may be involved in suppressing tics
(3–
5).
Early studies of brain morphology in individuals with Tourette’s disorder measured volumes of the basal ganglia. A deviation from the normal leftward predominance of basal ganglia volumes was reported in subjects with Tourette’s disorder
(6,
7). These findings suggested a disturbance of cerebral lateralization, a possibility that was further supported by reports of reduced behavioral lateralization in subjects with Tourette’s disorder
(8,
9). Because the corpus callosum helps to support the lateralization of brain function by segregating and integrating function across the cerebral hemispheres
(10), disturbances in corpus callosum morphology have been postulated to contribute to the presumed aberrant cerebral lateralization in subjects with Tourette’s disorder
(11).
The first investigation of the corpus callosum in persons with Tourette’s disorder
(12) reported a smaller overall cross-sectional area in young adults with Tourette’s disorder. A subsequent study of children and adolescents reported that a diagnosis of Tourette’s disorder (without comorbid attention deficit hyperactivity disorder [ADHD]) was associated with a larger anterior body of the corpus callosum, whereas a diagnosis of ADHD (without Tourette’s disorder) was associated with a smaller area of this same corpus callosum subregion
(13). Larger corpus callosum areas were reported in a subsequent study of adults with Tourette’s disorder
(14), and corpus callosum size was reported to be normal in girls with Tourette’s disorder
(15). Small sample sizes in previous studies, the inclusion of subjects of various ages that were often not well matched across study groups, and differing frequencies of comorbid illnesses all likely contributed to the variability in findings
(16–
20).
Imaging technologies have improved considerably since those earlier studies. Midsagittal images, for example, previously were not reformatted to correct for head tilt and rotation, and therefore they did not permit measurement of the corpus callosum at its true midline. Because interhemispheric fibers rapidly fan upward and downward as they cross the corpus callosum midline, measurements of the corpus callosum that do not correct for imperfect head positioning will capture corpus callosum subregions variably either at midline or off midline in the same subject and will thereby increase variability of measurement of the cross-sectional size of the corpus callosum in its midsagittal views
(21). Thick slices (typically 5 mm in earlier studies) added to this variability and likely increased the overall mean cross-sectional area, because more of the corpus callosum would have been captured within the thickness of the slice as fibers fanned out across the midline. Finally, correction of corpus callosum size for overall brain volume in those earlier studies was typically done by using intracranial area measured on a single sagittal slice, which is a crude estimate of overall brain size.
The corpus callosum is the major commissure connecting the homologous cortical regions across the cerebral hemispheres, and cortical regions are thought to be important in the pathophysiology of Tourette’s disorder. In large samples of subjects with Tourette’s disorder and comparison subjects, for example, dorsolateral prefrontal cortices were significantly larger in children with Tourette’s disorder but smaller in adults with Tourette’s disorder
(5,
22). Prefrontal volumes correlated inversely with the severity of tic symptoms, suggesting that the larger prefrontal volumes in children could represent adaptive processes that help to reduce tic severity
(3,
5). A functional magnetic resonance imaging (MRI) study, moreover, demonstrated that prefrontal cortices activate robustly during the willful suppression of tic behaviors
(4), further suggesting that hypertrophy of prefrontal cortices helps to reduce tic symptoms by improving inhibitory control over unwanted impulses and automatic behaviors, a function that the prefrontal cortex is generally believed to subserve
(23). Given the importance of prefrontal functioning in the pathophysiology of Tourette’s disorder and the dense interconnections of the corpus callosum with these cortical regions, the corpus callosum seems a likely region to influence tic symptoms in persons with Tourette’s disorder by influencing the functioning and interaction of prefrontal cortices across the cerebral midline.
In the current MRI study of 158 subjects with Tourette’s disorder and 121 healthy comparison subjects, we hypothesized that the overall cross-sectional areas of the corpus callosum would differ between subjects with Tourette’s disorder and healthy comparison subjects, reflecting altered cortical connectivity of the corpus callosum in the group with Tourette’s disorder. We furthermore hypothesized that the effects of Tourette’s disorder on corpus callosum size would depend on age, as has been found previously for both the cortex and basal ganglia
(5,
24). Post hoc analyses were used to assess the associations of corpus callosum size with the severity of tic symptoms and with the volumes of cortical subregions.
Method
Subjects
Patients were recruited from the Tic Disorder Clinic at the Yale Child Study Center and met the DSM-IV criteria for a diagnosis of Tourette’s disorder. Healthy comparison subjects were recruited randomly from a telemarketing list of 10,000 names of individuals who lived in the same ZIP code areas as the subjects with Tourette’s disorder. Written informed consent was obtained from all participants after the procedures were carefully explained. The human investigation committee at Yale School of Medicine, New Haven, Conn., approved the study.
Exclusion criteria for the comparison group included a lifetime history of tic disorder, obsessive-compulsive disorder (OCD), ADHD, psychotic disturbance, or any current axis I disorder. Additional exclusion criteria for both groups were epilepsy, history of head trauma with loss of consciousness, former or present substance abuse, and an IQ below 80. Diagnoses were established by using the Schedule for Tourette and Other Behavioral Syndromes
(25), which includes the Schedule for Affective Disorders and Schizophrenia for School-Age Children—Present and Lifetime Version
(26), and a best estimate consensus procedure that considered all available study materials
(27), including medical records. OCD symptoms were quantified with the Yale-Brown Obsessive Compulsive Scale
(28,
29). ADHD symptoms were assessed with the Conners Parent and Teacher Rating Scales
(30,
31), the Child Behavioral Checklist
(32), and the DuPaul-Barkley ADHD Rating Scale
(33,
34). The severity of tics was rated with the Yale Global Tic Severity Scale
(35). Socioeconomic status was estimated with the Hollingshead Four-Factor Index
(36). Interviews of family members and childhood medical histories helped to establish childhood and lifetime diagnoses for the adult subjects.
Included in the statistical analyses were 158 subjects with Tourette’s disorder and 121 healthy comparison subjects, ages 5–65 years (
Table 1). Seventy-four percent (N=117) of the group with Tourette’s disorder and 55% (N=67) of the comparison group were male. In the group with Tourette’s disorder, 32 individuals (20%) had combined-type ADHD, 39 (25%) had OCD, and 10 (6%) had both comorbidities in their lifetimes. At the time of the MRI scanning, 75 subjects (47.5%) in the group with Tourette’s disorder were taking medication, including traditional neuroleptics (N=12 [7.6%]), risperidone (N=4 [2.5%]), α agonists (N=22 [14.0%]), selective serotonin reuptake inhibitors (SSRIs) (N=12 [7.6%]), tricyclic antidepressants (N=9 [5.7%]), a combination of traditional neuroleptics with SSRIs (N=4 [2.5%]), or other combinations (N=11 [6.9%]). The comparison subjects took none of these medications.
The children with Tourette’s disorder had less severe motor tics than did the adults with Tourette’s disorder, both at the time of scanning (Yale Global Tic Severity Scale motor tic mean score=11.4, SD=4.6, versus mean=14.9, SD=4.2) (t=–4.2, df=149, p<0.001) and in their lifetimes (mean=15.0, SD=4.3, versus mean=18.8, SD=2.7) (t=–6.4, df=142, p<0.001). The children with Tourette’s disorder also had less severe worst-ever phonic tic scores than did the adults with the disorder (mean=11.6, SD=4.5, versus mean=14.4, SD=4.4) (t=–3.4, df=142, p<0.001).
MRI Scanning and Image Analysis
Head positioning was standardized by using canthomeatal landmarks. T
1-weighted, sagittal three-dimensional volume spoiled gradient echo images were acquired for all subjects (TR=24 msec, TE=5 msec, 45° flip angle, 256×192 matrix, field of view=30 cm, two excitations, frequency encoding superior/inferior, slice thickness=1.2 mm, 124 contiguous slices). Morphometric analyses were performed by using ANALYZE 7.5 software (Rochester, Minn.) on Sun Ultra 10 workstations (Sun Microsystems, Santa Clara, Calif.). Raters were blind to subject characteristics and group assignments. Each data set was realigned to the midsagittal slice by using standard midline landmarks (callosal sulcus, cerebral aqueduct, pineal gland, peaked roof of the fourth ventricle, and minimal gray matter in the interhemispheric fissure). Realignment minimizes variability in corpus callosum size between subjects that may have been caused by differences in head positioning within the scanner
(21).
Corpus callosum definition
The midsagittal slice was magnified eightfold. The corpus callosum contour was segmented automatically by using an isointensity contour function and was then manually edited. Its endpoints at the rostrum and splenium were identified by using local curvature maxima. The contour was divided into two segments (upper and lower) based on these endpoints, and each segment was sampled evenly to 100 points by using percentiles along the two curves. The corpus callosum centerline was constructed by using the midpoints of 100 line segments that connected the corresponding points at each percentile on the upper and lower segments of the corpus callosum contour. The centerline was then divided into five equal lengths by perpendicular chords spanning the corpus callosum width
(12,
37) (
Figure 1). The fifths of the corpus callosum centerline thus defined five corpus callosum subregions: genu/rostrum, anterior midbody, posterior midbody, isthmus, and splenium. In 20 scans measured blindly, the interrater intraclass correlation coefficients for these measures were >0.95.
Cerebral subdivisions
Methods for subdividing the cerebrum into dorsal prefrontal, inferior occipital, midtemporal, orbitofrontal, premotor, parieto-occipital, subgenual, and sensorimotor regions have been described previously
(5). Whole brain volume, used as a covariate in all statistical analyses to control for general scaling effects in the brain, was defined as all gray and white matter, together with the CSF of the cortical sulci, ventricles, and cisterns. CSF spaces were included to minimize the effects of cerebral atrophy in the brains of older subjects.
Statistical Analyses
Statistical analyses were performed with SPSS
(38) or R software
(39). All p values were two-sided.
Regression analysis
General linear regression was used to test our a priori hypotheses of diagnosis- and age-specific abnormalities in overall size of the corpus callosum in individuals with Tourette’s disorder. Overall area of the corpus callosum was entered as the dependent variable in the analysis. Diagnostic category was entered as an independent variable. Covariates included the lifetime diagnoses of ADHD or OCD, as well as sex, age, and whole brain volume. The square of age (age
2) was also included to accommodate a possible curvilinear effect of age on size of the corpus callosum
(37,
40). All two-way interactions of these variables were considered for inclusion in the model based on a backward, stepwise automatic selection of regression variables. All models were hierarchically well formulated, with the lower order terms contributing to significant interactions retained in the model and with all other nonsignificant interactions and variables eliminated from it. Corpus callosum measures were normally distributed.
Repeated-measures analysis of variance (ANOVA) mixed-effects model
In testing for the influence of diagnosis in different corpus callosum subregions, we used a mixed-effects model in S-Plus/R software
(41) to estimate the corpus callosum subregions. The within-subjects factor was “corpus callosum region” with five levels. The same covariates that were used in the univariate analyses were used in the repeated-measures model. Selected three-way interactions (e.g., Tourette’s disorder-by-age-by-region) were considered in the repeated-measures model.
Correlations with tic severity
Correlations of corpus callosum size with the severity of tic symptoms were assessed both for the time of scanning and for the time of the patient’s life when motor or vocal tics were most severe. These correlation analyses included whole brain volume and sex as covariates.
Correlations with cortical volumes
We assessed the correlations of regional cerebral volumes with the cross-sectional area of each corpus callosum subregion as indices of anatomical connectivity of cortical regions across these subregions. Scaling effects were controlled by partialing out whole brain volume and sex. Significant differences in magnitudes of the correlation coefficients between the group with Tourette’s disorder and the comparison group were tested by using the test statistic D
(42) for comparing two Pearson correlations, corrected for the degrees of freedom:
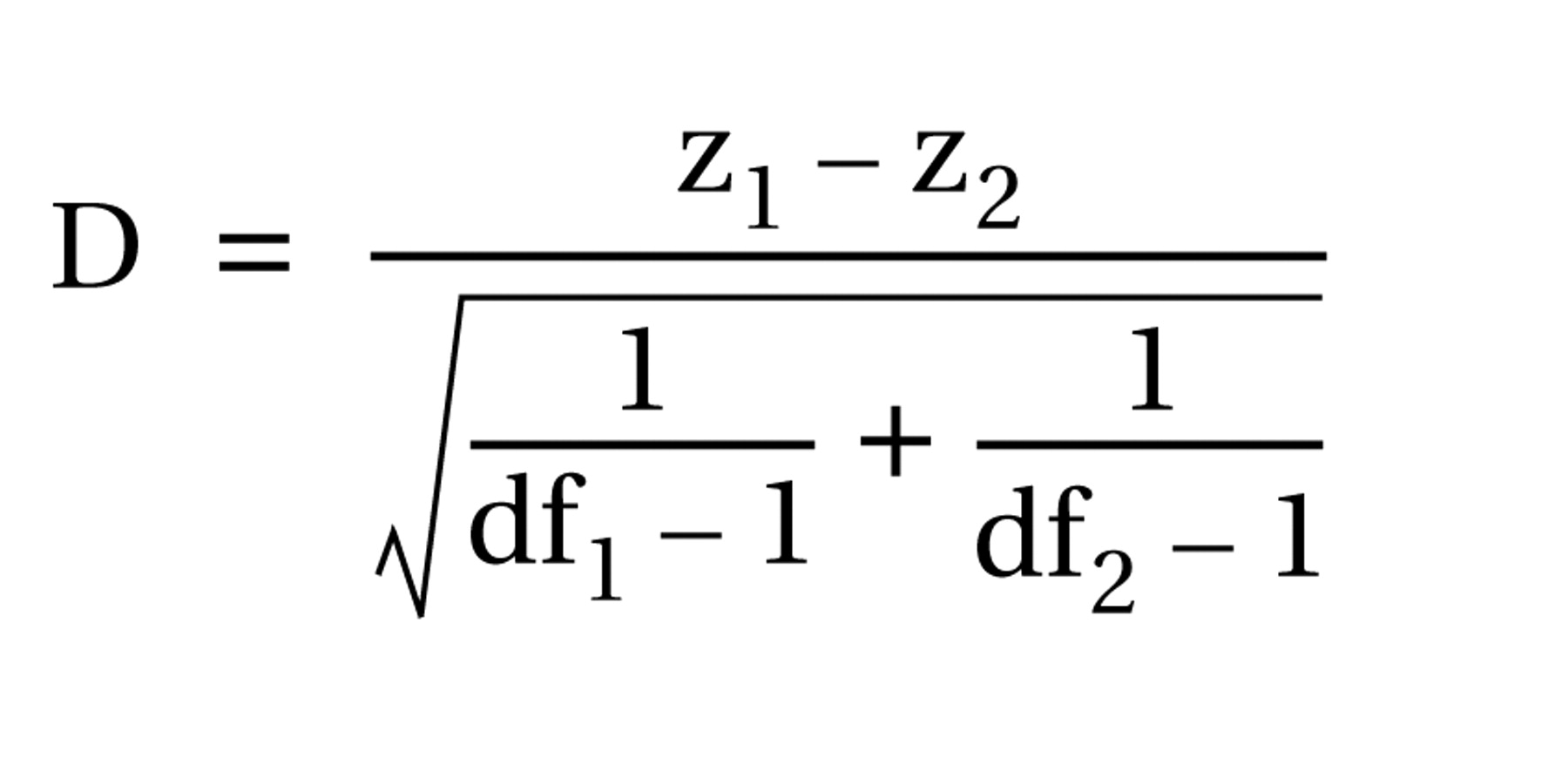
where
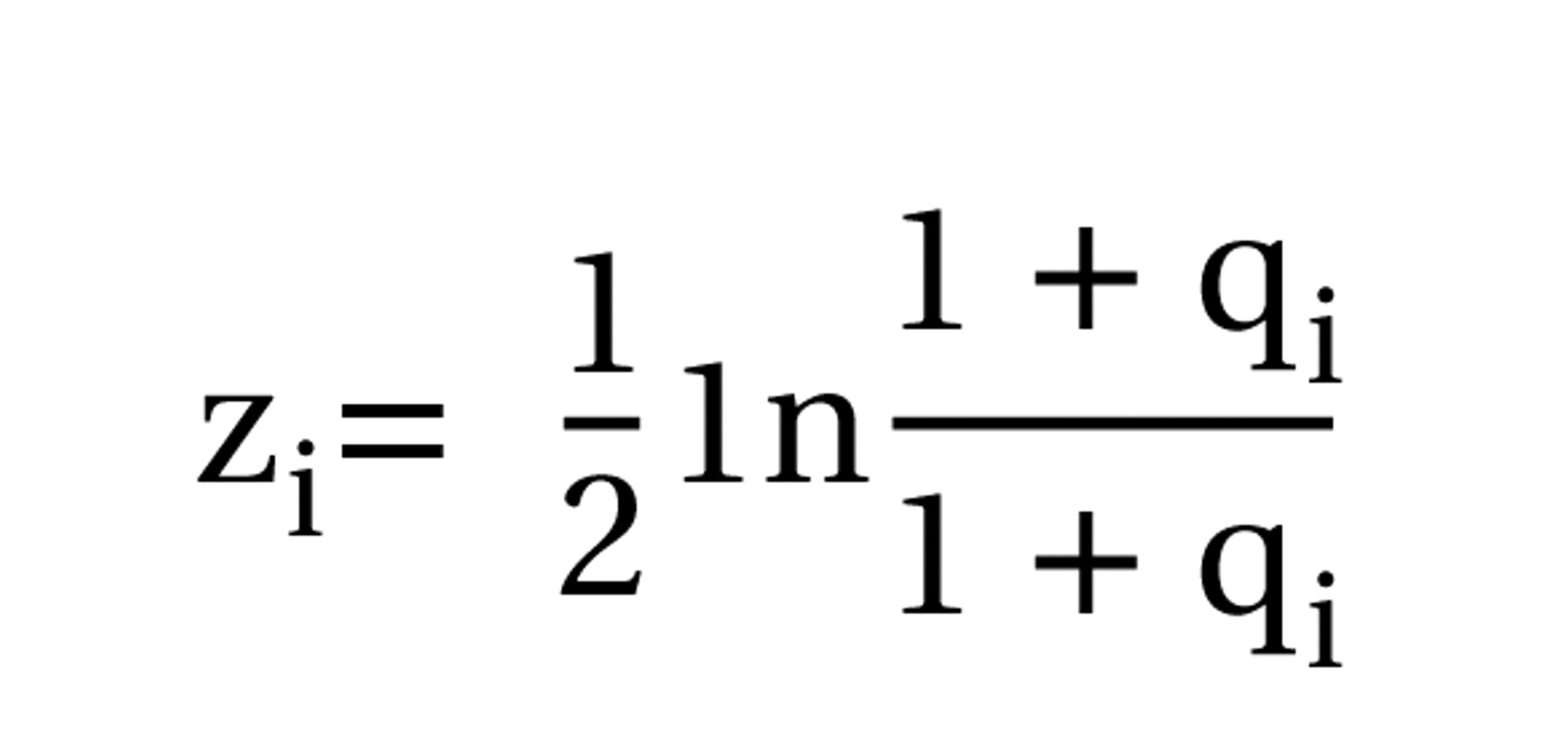
are the Fisher transforms of the correlation coefficients for two samples of sizes n
i and df
i (degrees of freedom)=ni–p–1 for partial correlations when p variables are partialed out (here p=2). The distributions of the transformed variables were assessed with nonparametric and semiparametric bootstrapping procedures
(43,
44) and found to be normal.
Handedness
Possible influences of handedness were assessed by testing the significance of this variable in the final statistical model.
Medication
Possible medication effects were assessed in two ways. First, the main effects of current medication use, dichotomously coded as 0 or 1, were tested separately in the final model for the classes of traditional neuroleptics (e.g., haloperidol or pimozide), risperidone, α agonists (clonidine or guanfacine), or specific SSRIs. Second, to ensure that neuroleptic use was not affecting the findings for modeling of diagnostic effects in the entire subject group, the statistical model was rebuilt when the 27 individuals who were taking either typical or atypical neuroleptics were excluded, resulting in N of 131 for the group with Tourette’s disorder and N of 121 for the comparison group.
Comorbid conditions
The effects of ADHD and OCD comorbidity were controlled statistically by including these diagnoses as main effects and interactions in the statistical models. To ensure further that these effects were not influencing the findings for the diagnostic effects of Tourette’s disorder on corpus callosum size in the entire study group, the analysis was repeated while excluding all individuals with Tourette’s disorder who had a lifetime diagnosis of OCD or ADHD, leaving only subjects with “pure” Tourette’s disorder (N=77) and the comparison subjects (N=121).
Results
Univariate Regression Analyses
A priori hypothesis testing
A diagnosis of Tourette’s disorder was significantly associated with a smaller overall corpus callosum area (t=–2.9, df=270, p<0.005). Interpretation of this effect of diagnosis was complicated, however, by the presence of a strong interaction of diagnosis with age (t=3.6, df=270, p<0.001), which indicated that the smaller overall size of the corpus callosum was attributable to the larger proportion of children with Tourette’s disorder in this study, as they had smaller mean callosal area (mean=492.23 mm
2, SE=7.16) than the children in the comparison group (mean=507.40 mm
2, SE=9.35) (
Figure 2). Adults with Tourette’s disorder, in contrast, had larger mean area of the corpus callosum (mean=580.16 mm
2, SE=11.40), relative to same-age comparison subjects (mean=541.55 mm
2, SE=10.06). This age-by-diagnosis interaction, which explained the greatest proportion of variance in corpus callosum size, confirmed our second a priori hypothesis.
The mixed-effects, repeated-measures analysis of variance
(41) did not yield a significant interaction of region with diagnosis, indicating that the effect of diagnosis on corpus callosum size was not specific to particular corpus callosum subregions. Moreover, no significant Tourette’s disorder-by-age-by-region effect was detected, indicating that the age-related diagnostic effects did not appreciably vary by corpus callosum subregions. These findings indicate that group differences in corpus callosum size tended to generalize across all corpus callosum subregions.
Other effects
The Tourette’s disorder-by-whole-brain-volume interaction was significantly associated with size of the overall corpus callosum (t=2.4, df=270, p<0.02). Further probing of the interaction revealed that a smaller whole brain volume in the Tourette’s disorder group was accompanied by a disproportionately smaller corpus callosum, whereas a larger whole brain volume was accompanied by a disproportionately larger corpus callosum. In addition, older age (t=5.6, df=270, p<0.0001), whole brain volume (t=3.1, df=270, p<0.002), and male sex (t=3.4, df=270, p<0.001) were strong independent predictors of larger overall corpus callosum size. Age2 was significantly inversely associated with corpus callosum area (t=–4.2, df=270, p<0.0001). The age-by-sex interaction was also a strong predictor of corpus callosum size (t=–3.9, df=270, p<0.001), showing a steeper correlation with age in the female group. No significant influence of handedness was detected.
Medication effects
Medication effects were assessed in all final statistical models, and no relevant effects were observed. Furthermore, when data for the individuals who were taking neuroleptic medication were excluded, the findings did not differ appreciably from those obtained in the analysis for the entire study group. Therefore, medication use did not seem to influence findings in any discernible way.
Effects of comorbid illnesses
We could not detect an influence of comorbid OCD, comorbid ADHD, or any of the interactions with OCD or ADHD on corpus callosum size.
When data for all individuals with comorbid OCD or ADHD were excluded, a diagnosis of Tourette’s disorder still was associated significantly with a smaller overall corpus callosum area (t=–2.2, df=198, p<0.03) and with a prominent interaction of a diagnosis of Tourette’s disorder with age (t=2.8, df=198, p<0.007). Therefore, for these subjects with “pure” Tourette’s disorder, the findings were similar to those reported for the entire study group.
Correlation Analyses
Tic severity
After adjustment for whole brain volume and sex, corpus callosum size correlated significantly and positively with severity of motor tics (both current and worst ever) (
Table 2) but not with severity of phonic tics.
Cortical volumes
Partial Pearson correlations of cortical volumes with corpus callosum size were prominent in the group with Tourette’s disorder and the comparison group, particularly for overall corpus callosum size, which correlated inversely with volumes of the dorsolateral prefrontal cortex (subjects with Tourette’s disorder: r=–0.49, p<0.001, N=151; comparison subjects: r=–0.25, p<0.01, N=117) and orbitofrontal cortex (subjects with Tourette’s disorder: r=–0.23, p<0.005, N=151; comparison subjects: r=–0.03, p=0.80, N=117). Positive correlations were observed for premotor (subjects with Tourette’s disorder: r=0.41, p<0.001, N=153; comparison subjects: r=0.30, p<0.001, N=121) and sensorimotor cortices (subjects with Tourette’s disorder: r=0.19, p<0.02, N=153; comparison subjects: r=0.13, p=0.15, N=121). The inverse correlations of corpus callosum area with frontal volumes were strongest for the most anterior portions of the corpus callosum in both groups. The inverse correlations of dorsolateral prefrontal cortex volumes with corpus callosum size were significantly stronger in the group with Tourette’s disorder than in the comparison group for the total corpus callosum, genu/rostrum, and splenium areas (
Table 3).
Discussion
The major findings of this study are that younger individuals with Tourette’s disorder had smaller overall corpus callosum size, relative to healthy comparison subjects, that the reductions in corpus callosum size in addition depended on the age of the subject (smaller corpus callosum sizes were detected in children with Tourette’s disorder and larger corpus callosum sizes were found in adults), and that corpus callosum size correlated positively with the severity of tic symptoms. The findings remained unchanged when data for individuals who were taking neuroleptics and individuals who had comorbid illnesses (ADHD or OCD) were excluded in separate analyses.
We cannot definitively infer causation from these cross-sectional, correlational findings
(45,
46), which can be interpreted to mean either that disturbances in corpus callosum size contribute to the genesis of tics, that they are a consequence of having tics, or that they represent a downstream, epiphenomenal effect of disturbances elsewhere in the brain. Nevertheless, compelling post hoc findings suggest that the disturbances of corpus callosum morphology in Tourette’s disorder likely do not contribute to the genesis of tics but rather contribute to a compensatory response within prefrontal cortical regions that helps to modulate the severity of tic symptoms. These post hoc findings include the correlation of age with size of the corpus callosum and the correlations of corpus callosum size with both symptom severity and volumes of prefrontal cortices.
Age Effects
In both the group with Tourette’s disorder and the comparison group, age accounted for most of the variance in overall size of the corpus callosum. Corpus callosum size increased steeply with age until approximately 30 years of age, after which it steadily declined. These correlations of corpus callosum size with age differed across diagnostic groups (represented statistically in a significant diagnosis-by-age interaction). Whereas we previously found that volumes of the prefrontal cortex were larger in children and smaller in adults with Tourette’s disorder than in healthy comparison subjects, in the current study corpus callosum sizes were smaller in children with Tourette’s disorder and larger in adults with Tourette’s disorder. Thus, the directions of group differences in the corpus callosum and prefrontal cortex were diametrically opposite one another for each age group, demonstrating conclusively that the corpus callosum findings in subjects with Tourette’s disorder do not simply represent an extension to the cerebral midline of the findings reported for the prefrontal cortex.
The children and adults with Tourette’s disorder in this study likely represent differing subpopulations of all individuals who have a lifetime diagnosis of Tourette’s disorder. Tic symptoms in most individuals with Tourette’s disorder either remit or improve substantially by young adulthood
(47), and the adults in this study (as in all previous imaging studies of Tourette’s disorder) were still highly symptomatic. The disease process in adults with persistent tics likely differs from that in most patients with Tourette’s disorder in ways that have not yet been fully understood. The larger corpus callosum detected in adults with Tourette’s disorder could therefore represent a morphological feature associated with the continuation of tic symptoms into adulthood and the failure of tic symptoms to remit in late adolescence
(3,
47).
Correlations With Tic Severity
The association of corpus callosum size with the severity of tic symptoms supports the possibility that the smaller corpus callosum size in children with Tourette’s disorder serves a compensatory function. After the effects of brain size and other demographic characteristics were controlled, overall corpus callosum size and the sizes of corpus callosum subregions correlated positively with the severity of motor tics. In other words, less severe tics accompanied smaller corpus callosum size in this group of subjects with Tourette’s disorder. Because these associations with tic severity were detected both in the entire group with Tourette’s disorder and in the subgroup of children with Tourette’s disorder, the findings were not driven by the inclusion of adults with Tourette’s disorder. We speculate that smaller corpus callosum sizes may be instrumental in reducing the severity of tic symptoms, possibly by reducing neuronal trafficking between prefrontal cortices across the cerebral midline
(48,
49).
Although the neurotransmitter specifications of most transcallosal axons are excitatory
(50–
52), experimental evidence suggests that the corpus callosum in vivo has powerful inhibitory functions within the cerebral cortex, functions that are presumably mediated by synapses of corpus callosum projections onto γ-aminobutyric acid (GABA)-ergic inhibitory interneurons within cortical gray matter
(53–
55). Thus, a smaller corpus callosum in Tourette’s disorder may help to modulate the severity of tics by reducing excitation across the cerebral hemispheres to these GABA-ergic interneurons, thereby reducing the net inhibition and enhancing excitation of the prefrontal regions that help to control tic behaviors
(4). This hypothesis is consistent with the previous suggestion that the cortical hyperexcitability reported previously in Tourette’s disorder
(56) may be caused by the reduced activity of cortical inhibitory interneurons
(1).
Correlations With Cortical Volumes
In both the healthy groups and the group with Tourette’s disorder, corpus callosum size correlated inversely with the volumes of prefrontal regions and positively with the volumes of premotor regions. These correlations were most significant for anterior subregions of the corpus callosum, consistent with the known topography of interhemispheric projections through corpus callosum subregions
(57,
58). It is important to note that the magnitudes of these correlation coefficients were significantly stronger for the group with Tourette’s disorder than for the healthy comparison subjects. The greater magnitude of the correlations with prefrontal volumes in the group with Tourette’s disorder suggests the possibility that the opposing diagnostic effects in the prefrontal cortex (larger in children with Tourette’s disorder) and in anterior corpus callosum subregions (smaller in children with Tourette’s disorder) may have been caused by an exaggeration of the normal physiological processes that created the inverse correlation between the sizes of the anterior corpus callosum regions and prefrontal cortices in the healthy subjects. The correlations of corpus callosum size with symptom severity (smaller size accompanied less severe tics), the age-specific effects in subjects with Tourette’s disorder (larger size was associated with the failure of symptoms to remit), and the hypothesized functions of larger prefrontal cortices (the suppression of tic symptoms) suggest that these exaggerated correlations between the size of the anterior corpus callosum and the volumes of prefrontal cortices may represent compensatory responses that help to attenuate the severity of tic symptoms.
This hypothesized co-opting of normal developmental processes for compensatory purposes would constitute developmental neural plasticity in children with Tourette’s disorder. Plasticity within the human central nervous system is well documented
(59–
64). Moreover, the widespread and transient overproduction of callosal projections may make the corpus callosum particularly amenable to plastic remodeling in response to experiential demands
(50,
65,
66). In humans, pruning and myelination of the corpus callosum coincide with cortical maturation, suggesting that development of the corpus callosum and the cerebral cortex mutually influence one another
(67,
68). Experience-dependent plasticity of the corpus callosum has been suggested by the reported correlation of corpus callosum size with the duration of skill training
(69) and with the presence of pathological conditions
(70,
71) whose etiologies may depend on the quality of perceptual experience during sensitive periods of development.
Scaling Effects
A significant interaction of diagnosis with brain size was detected for the overall size of the corpus callosum. This effect derived from smaller than expected corpus callosum sizes in subjects with Tourette’s disorder who had smaller than average brains and from larger than expected corpus callosum sizes in subjects with Tourette’s disorder who had larger than average brains. As yet, the biological significance of this finding is unknown.
Effects of ADHD and OCD Comorbidity
Although earlier studies have reported smaller rostral portions of the corpus callosum in children with ADHD
(16,
17,
72) and larger corpus callosum sizes in adults
(73) and children
(19) with OCD, we detected no evidence for an association of corpus callosum size with a diagnosis of ADHD or OCD comorbidity in this group of subjects. The subjects in our study were individuals with Tourette’s disorder, however, and the prior studies of ADHD or OCD included few if any subjects with this diagnosis. Thus, differences in findings are likely attributable to differences in the subject populations.
Limitations
As already noted, the presence of continuing tic symptoms in the adults with Tourette’s disorder in this study suggests that they were not representative of all subjects who have a lifetime diagnosis of Tourette’s disorder. Thus, the children with Tourette’s disorder were likely to be more representative of the larger population of clinically ascertained children with Tourette’s disorder, and our findings in the children are likely to provide a more representative understanding of the role of the corpus callosum in the pathophysiology of Tourette’s disorder than are our findings in adults with Tourette’s disorder. The age specificity of our findings underscores the difficulty of interpreting age correlations in a cross-sectional study
(45). Longitudinal studies are required for better understanding of the role of the corpus callosum in Tourette’s disorder throughout development.
In addition, the ultrastructural determinants of group differences and age correlates of the corpus callosum are unknown. The smaller size of the corpus callosum in children with Tourette’s disorder, for example, could represent a smaller number or less thickness of axons, less myelination or extracellular space, or smaller numbers of supporting glial cells, such as oligodendroglia or astrocytes
(74,
75). Postmortem and in vivo studies using other imaging modalities, such as magnetic resonance spectroscopy or diffusion tensor imaging, are needed to identify these ultrastructural determinants. Finally, we cannot entirely discount the possibility that medications contributed to our findings in the group with Tourette’s disorder, although we did not observe medication effects either in statistical modeling of those effects or in analyses of subgroups of medication-free subjects.
Conclusions
The present findings suggest that smaller corpus callosum size in individuals with Tourette’s disorder is a compensatory, neuroplastic response to the presence of tic symptoms that enhances prefrontal functioning and thereby better controls subjects’ tic behaviors through reduced interhemispheric inhibition of prefrontal control. Longitudinal studies are required to test fully the validity of this interpretation of our findings. In particular, correlations of changes in size of the corpus callosum with changes in prefrontal volumes and with changes in tic severity should be assessed in children with Tourette’s disorder as they age. Future studies should include use of diffusion tensor imaging to study directly the corpus callosum fiber tracts that connect prefrontal regions across the hemispheres and use of electroencephalographic coherence patterns
(48) to study functional connectivity of prefrontal cortices across the midline. Transcranial magnetic stimulation would also be useful to assess transcallosal inhibitory influences of the corpus callosum on contralateral cerebral hemispheres
(76,
77).