A Molecular Signature of Depression in the Amygdala
Abstract
Method
Subjects
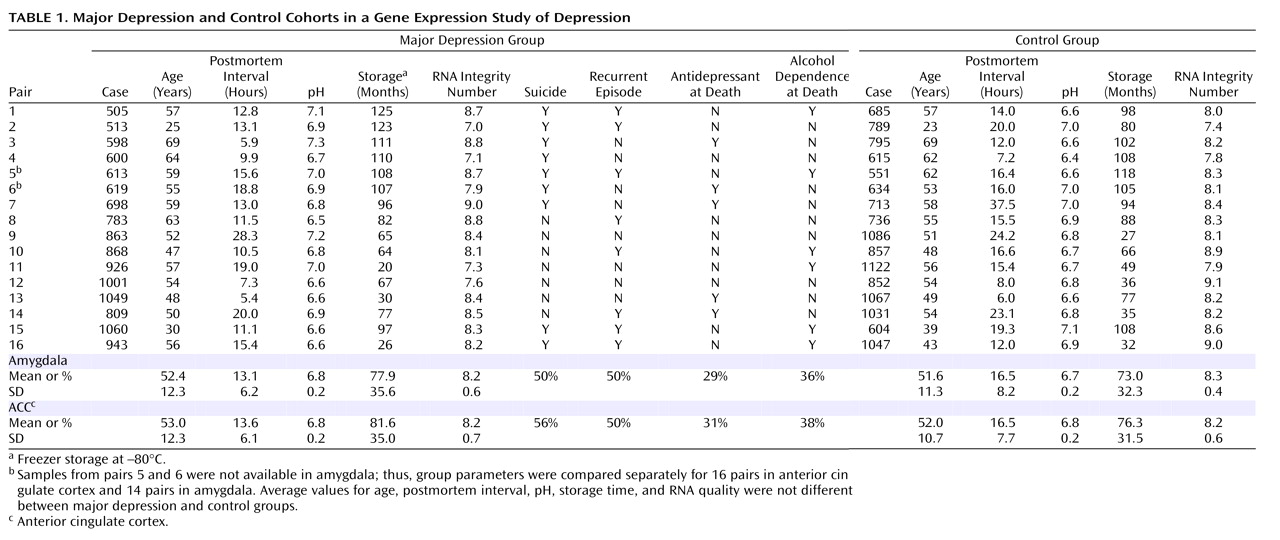
Brain Samples
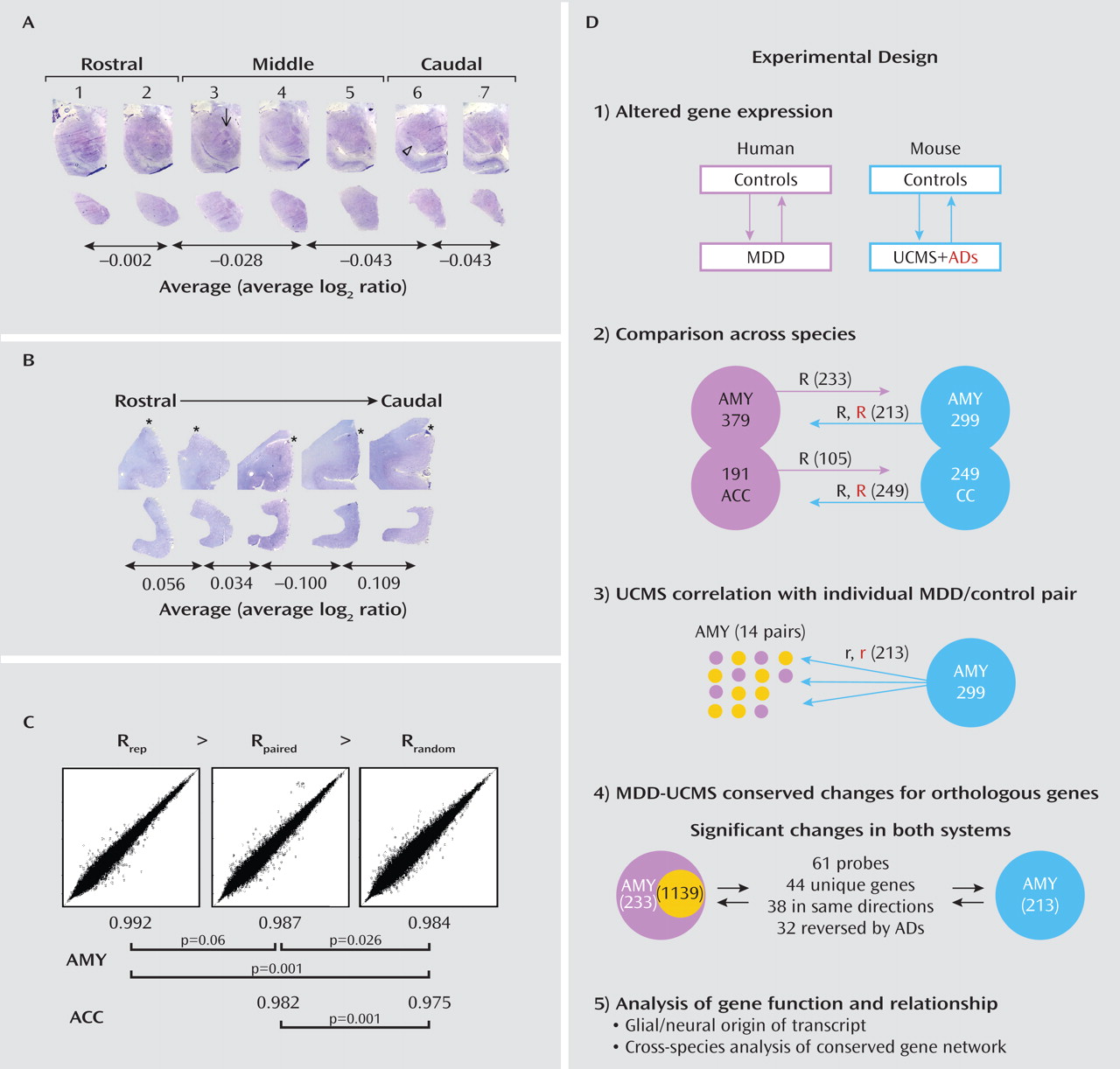
Microarray Samples
Microarray Quality Control
Rodent UCMS Model
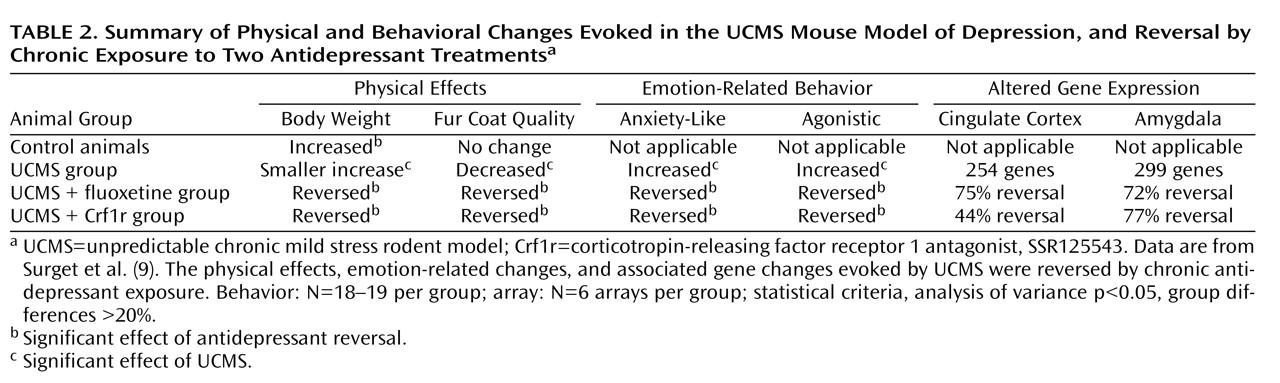
White Matter/Gray Matter Analysis
Array Data Statistical Analysis
Selection of significant genes
Statistical criteria
Real-Time qPCR
Western Blot Analysis
Gene Coexpression Networks
Results
Amygdala-Anterior Cingulate Cortex Altered Gene Expression in Human Major Depression
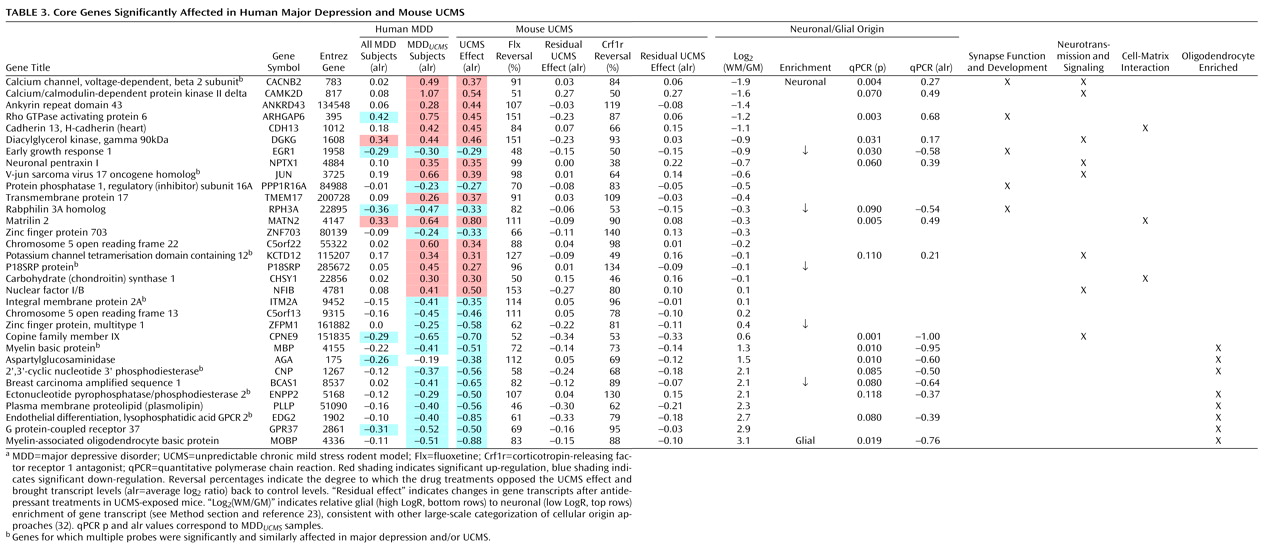
Profiles of Altered Gene Transcripts Are Conserved Between Human Major Depression and the UCMS Mouse Model of Depression and Are Reversed by Antidepressant Treatments in Mice
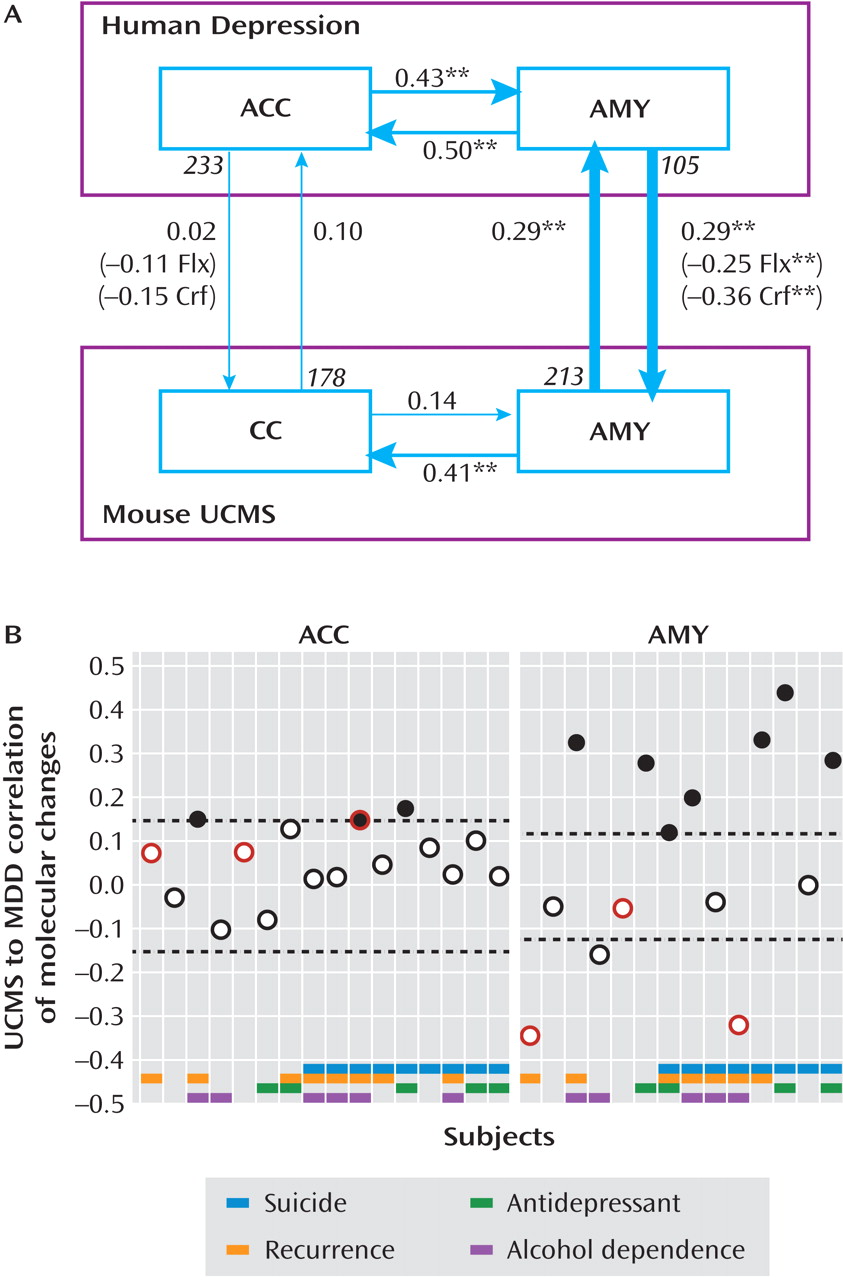
Amygdala Cross-Species Correlations of Depression-Related Molecular Changes Identified a Subgroup of Human Major Depression Subjects
Two Distinct Oligodendrocyte and Neuronal Depression-Related Phenotypes in Amygdala
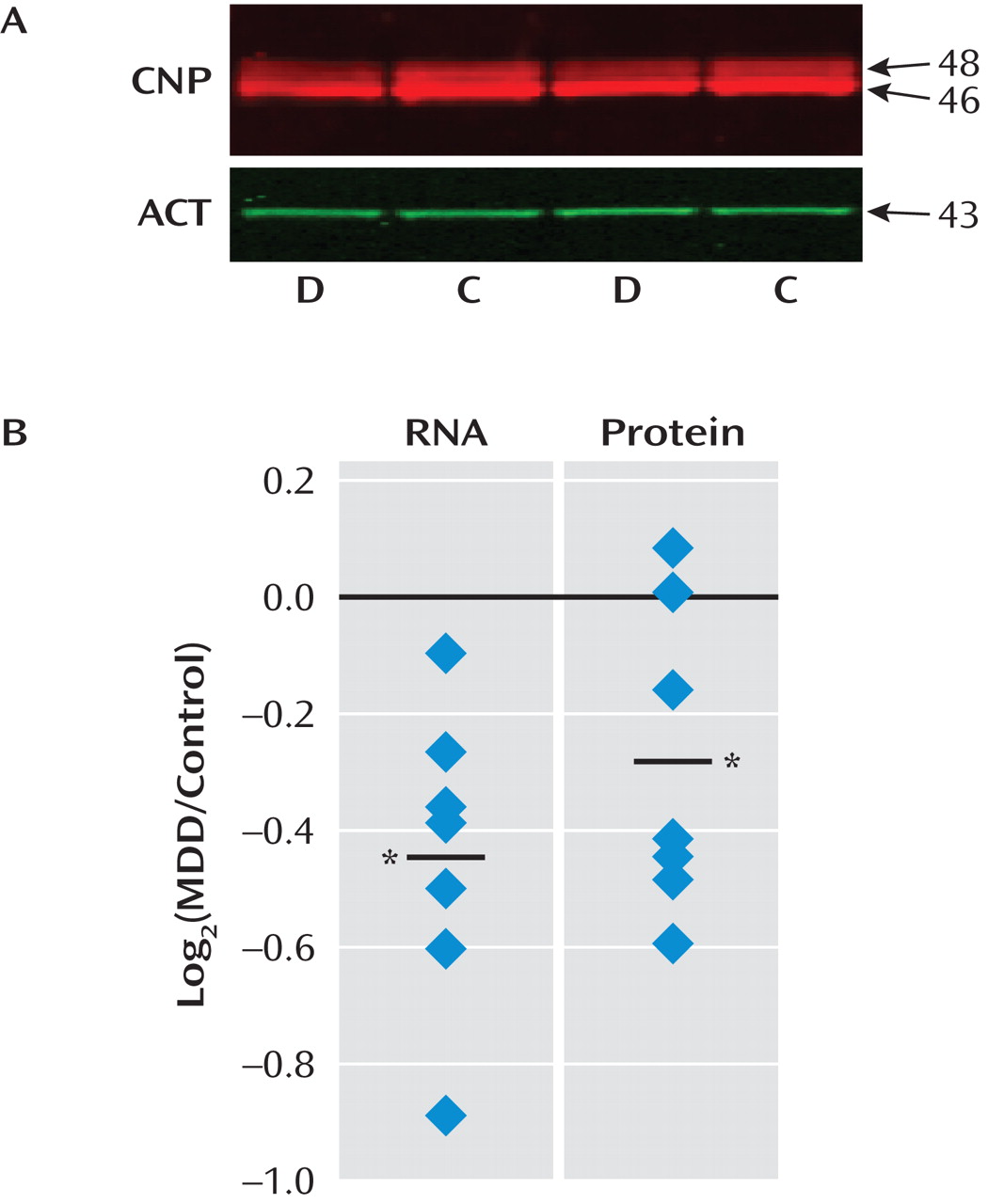
Genes With Conserved Major Depression- and UCMS-Related Changes Participate in a Highly Cohesive and Interactive Gene Coexpression Network
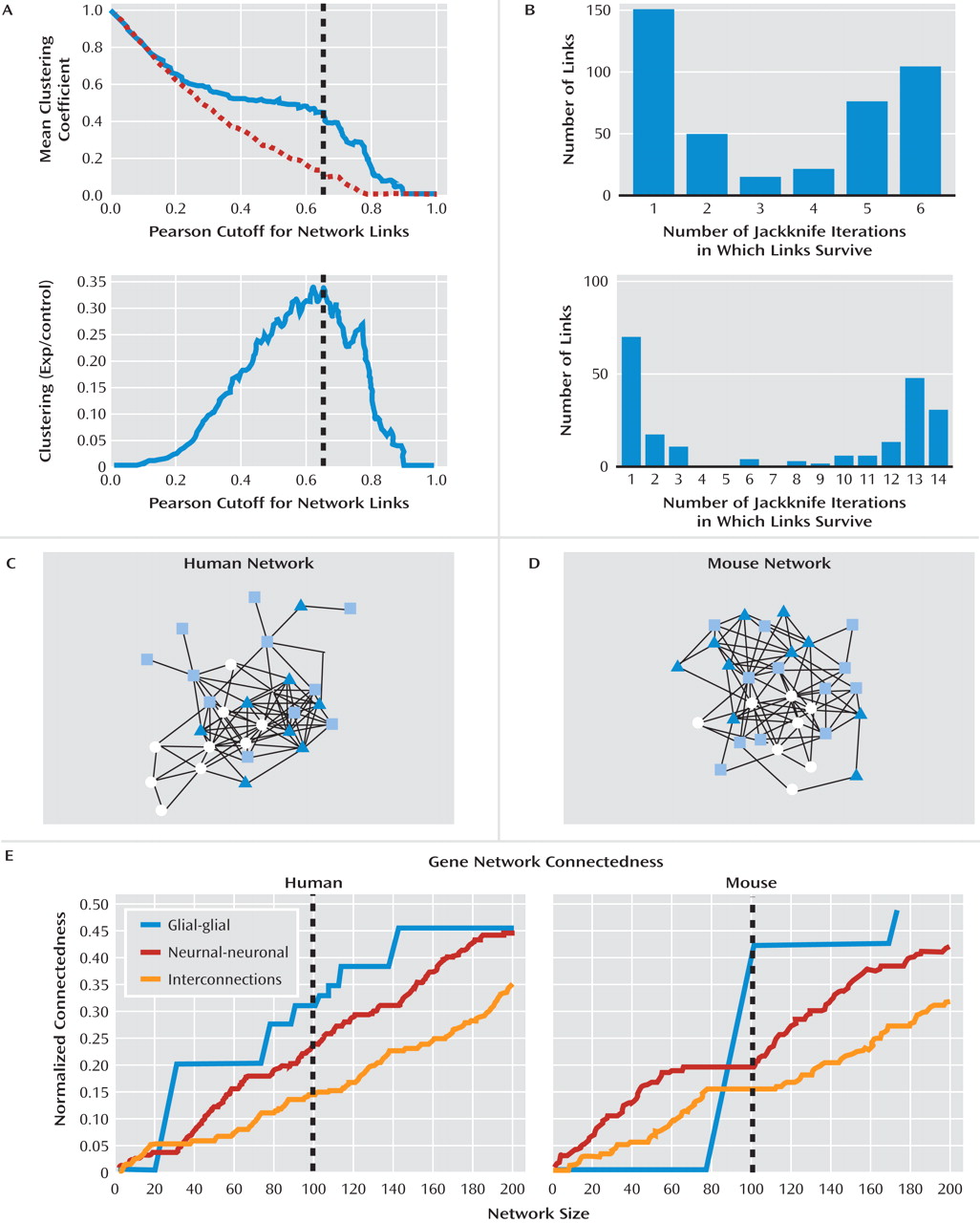
Discussion
A Phylogenetically Conserved Molecular Subtype of Depression in Amygdala
Oligodendrocyte and Principal Pyramidal Neuronal Changes in Amygdala in Depression
Footnotes
References
Information & Authors
Information
Published In
History
Authors
Metrics & Citations
Metrics
Citations
Export Citations
If you have the appropriate software installed, you can download article citation data to the citation manager of your choice. Simply select your manager software from the list below and click Download.
For more information or tips please see 'Downloading to a citation manager' in the Help menu.
View Options
View options
PDF/EPUB
View PDF/EPUBGet Access
Login options
Already a subscriber? Access your subscription through your login credentials or your institution for full access to this article.
Personal login Institutional Login Open Athens loginNot a subscriber?
PsychiatryOnline subscription options offer access to the DSM-5-TR® library, books, journals, CME, and patient resources. This all-in-one virtual library provides psychiatrists and mental health professionals with key resources for diagnosis, treatment, research, and professional development.
Need more help? PsychiatryOnline Customer Service may be reached by emailing [email protected] or by calling 800-368-5777 (in the U.S.) or 703-907-7322 (outside the U.S.).